Circadian clocks are internal molecular time-keeping mechanisms that enable organisms to adjust their behavior and physiology to the 24-h environment. In addition to the circadian system, the stress system effectively restores the internal dynamic equilibrium of living organisms, called homeostasis, in light of any threatening stimulus (i.e., stressor). Any dysregulation in either system or disturbance of their molecular interrelation might lead to severe health effects. Importantly, both systems communicate with and feedback on each other at various physiological and neuronal levels. Thus, any disturbance or uncoupling possibly contributes to the development of several somatic and affective disorders. In this chapter, we discuss the biological function of the circadian and the stress system, their interactions, and the clinical implications of their uncoupling or dysregulation. For the sake of clarity and focus, we will only address the mammalian central circadian clock in the brain.
Introduction
All inhabitants of our planet are exposed to recurrent environmental changes generated by the rotation of the earth in the solar system. In order to anticipate these predictable fluctuations, organisms have evolved an evolutionary conserved internal time-keeping system, i.e., the circadian clock. In mammals, the circadian system is organized in a hierarchical fashion: a central pacemaker is located in the bilateral suprachiasmatic nucleus (SCN) of the hypothalamus, whereas subsidiary peripheral clocks exist in virtually all tissues and organs (see Fig. 38.1) (for review see Ref. 7). Importantly, most species are also exposed to unpredictable changes in the environment, such as decreased food resources, increased predator numbers, or sudden changes in social states. To adapt to these sudden alterations, the acute activation of the stress response system comprising the hypothalamic-pituitary-adrenal (HPA) axis and the sympathetic nervous system (SNS) represents a fundamental survival mechanism. In contrast, chronic activation of the stress system might result in severe somatic and affective disorders (for review see Ref. 5). At first glance, the circadian and the stress system seem to represent two separate bodily control systems that are involved in adaptation to predictable and unpredictable stimuli, respectively. However, both systems are fundamental for survival and, thus, communicate with each other at various physiological and neuronal levels.
The central molecular clock
The mammalian SCN is formed by a heterogeneous bilateral network of 10,000–50,000 single neurons14 and serves as a master pacemaker in the control of a wide array of behavioral and physiological rhythms (e.g., sleep-wake, locomotion, cardiovascular function, endocrine processes) (see Box 38.1 and Fig. 38.1) (for review see Ref. 7). According to peptide expression, projection patterns, and neuronal morphology, each unilateral SCN can be divided into two subregions: a dorsomedial shell and a ventrolateral core region. Shell neurons produce primarily arginine-vasopressin (AVP) and are mainly innervated by limbic areas, the hypothalamus, and the core region. In contrast, vasoactive intestinal peptide (VIP) is mainly synthesized in the core region that receives most of the input from the retina and brain regions that receive photic input.32

HOW DO WE KNOW THAT THE SCN CONTROLS THE DAILY GC RHYTHM?
A series of electrical lesion studies in rodents in the 1970s provided clear evidence that the SCN possesses a primary role in the generation of mammalian circadian rhythms. Following selective disruption of the master SCN clock, locomotor activity, drinking behavior, body temperature, and GC secretion was completely absent.31,44 Despite the lack of neuronal connections between the grafted SCN and the host brain, transplantation of donor SCN tissue into hosts with lesioned SCN remarkably restored these rhythms, suggesting that a diffusible secreted factor might be responsible for transmitting the circadian signal from the SCN.40 However, while circadian rhythms of locomotor activity, drinking behavior, and body temperature could be restored by SCN transplants, circadian GC secretion was not, implicating that, in addition to secreted factors, neuronal efferents are required for generation of certain circadian rhythms.30
The Molecular Clockwork
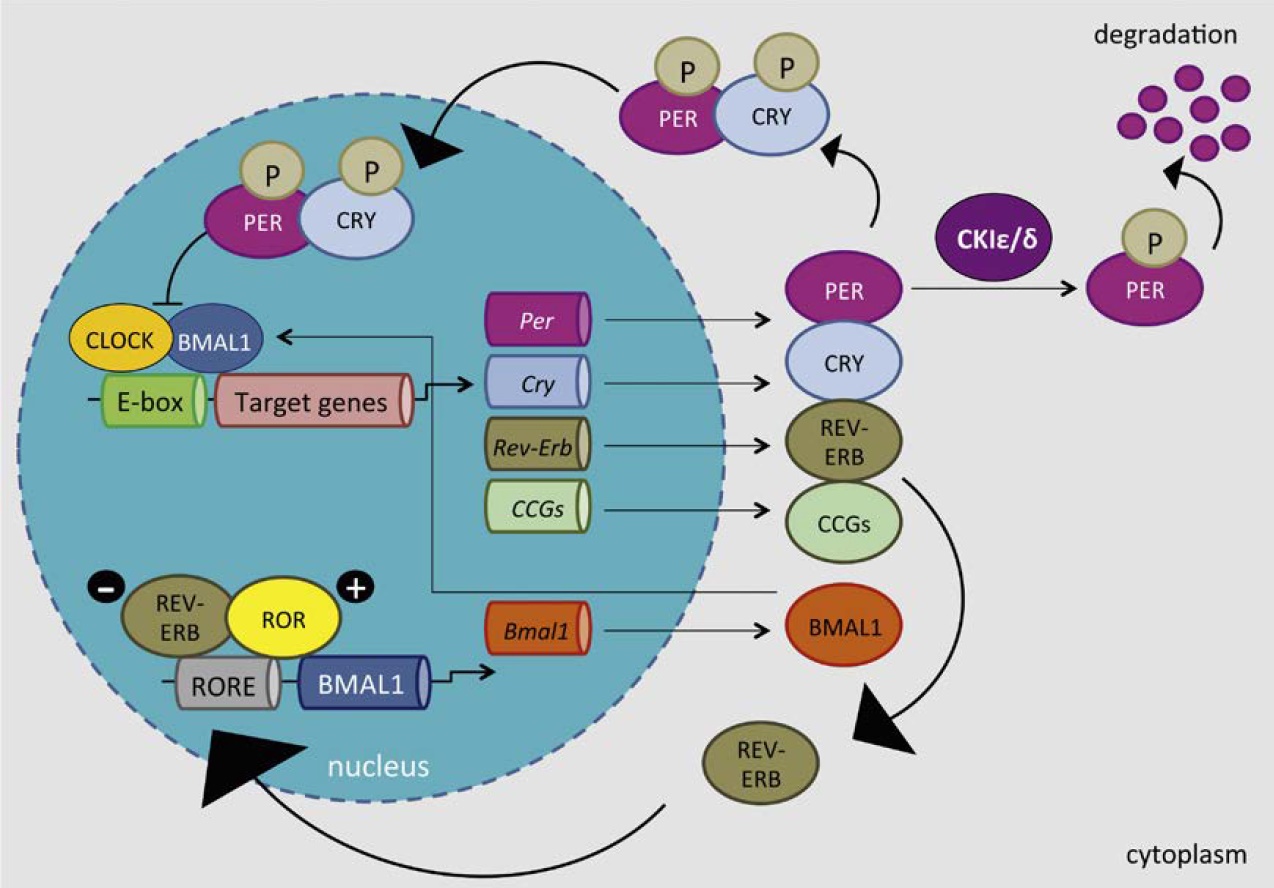
KEY POINTS
- => The circadian system is our control system for predictable environmental changes (e.g., day/ night, seasons). It consists of the central clock in the hypothalamic suprachiasmatic nucleus (SCN) and subsidiary peripheral clocks
-
=> The stress system is our control system for unforeseen changes in the environment (e.g., predators). It comprises the hypothalamicpituitary- adrenal (HPA) axis and the sympathetic nervous system (SNS)
-
=> Both systems are fundamental for survival and communicate with each other at various levels; disruptions in either system can lead to somatic and affective disorders
-
=> The central circadian clock activates the HPA axis, thereby controlling the daily glucocorticoid release from the adrenal cortex
-
=> Acute and chronic stress can affect core clock components within the SCN; disruptions of the molecular circadian clockwork is often related to depressive-like behavior
Stress

Sites of interaction between the circadian clock system and the stress system
In addition to the aforementioned stress-related secretion of GCs, these cholesterol-derived molecules are further released in a circadian manner under nonstressed conditions with an increased concentration prior to the active period of the day (early morning in humans, early evening in rats and mice).4,47 Furthermore, the circadian pattern is overlaid by an ultradian rhythm, i.e., a rhythm with a period significantly shorter than 24 h, with a pulse frequency (the rate of hormonal release) averaging between 60 and 90 min.47,48 The daily GC rhythm is controlled by the central circadian clock in a multimodal fashion: (1) the SCN controls the activity of the HPA axis by conveying excitatory and inhibitory information through synaptic contacts to the medioparvocellular PVN, where the CRH- and AVPexpressing neurons are located18; (2) the clock control over GC secretion operates via the sympathetic input to the adrenal gland, thereby modulating the sensitivity of the target organ to the incoming ACTH message35,45; and(3) the peripheral autonomous adrenal clock itself confers rhythmic expression of several clock genes as well as encoding molecules involved in the steroidogenic pathway and the ACTH signal transduction cascade in the zona glomerulosa and zona fasciculata of the adrenal gland. Thereby, mechanisms (2) and (3) are the main routes of SCN control over GC secretion. However, each of these paths might dominate under different conditions. The adrenal clockwork, for instance, appears to be essential for circadian GC production in mice in constant darkness, while under normal light–dark conditions ligh information is capable of regulating daily changes in GC production even in the absence of a functional local adrenal clock.43
The ultradian pulses that underlie the circadian GC rhythm occur with a relatively constant, roughly hourly, frequency, whereas the pulse amplitude (the amount of hormonal release) is variable. Besides the circadian input, homeostatic as well as stress-related signals impact the amplitude of these secretory episodes. The rising GC levels at the beginning of the active phase result from increases in the amplitude of the pulses, reaching its maximum just before awakening and declining thereafter to reach a trough early in the sleep phase (for review see Ref. 19). It has been demonstrated that the pulsatility of GC secretion is crucial for the appropriate stress response. The time of stressor application, for instance, determines the physiological stress response depending on the phase of an endogenous basal pulse. Rats that were exposed to white noise for 10 min responded with additional GC secretion if endogenous basal GC levels increased just prior to stressor exposure. In contrast, no or neglected GC responses were noticeable when basal endogenous GC levels were falling at stressor initiation. 48 These findings suggest that the basal GC pulsatility dynamically interacts with the ability of an organism to mount a stress response. Termination of the rising phase of the GC pulse by rapid feedback inhibition via the GR and MR may be essential in the generation of such pulses. While the GR mediates the acute effects of GC, the MR has an approximately 10-fold higher affinity than the GR and is involved in permissive or long-term activation during the peak of circadian GC concentration (for review see Ref. 21).
Circadian Responsiveness of the Hypothalamic-Pituitary-Adrenal Axis to Stress
Besides the commonly accepted interaction of the stress response and the time of day of stressor exposure in relation to psychological and physical stressors, recent studies suggest that this also applies for psychosocial stressors such as social defeat. Exposure of mice to 19 days of social defeat at the beginning of the active phase at Zeitgeber time (ZT)13–15 (ZT0 is defined as the time when lights are turned on) results in a more negative outcome (decreased social preference, reduced home–cage activity during the dark phase, more severe chemically induced colitis effects) as compared to stressor exposure at ZT1–3.2 In contrast, stress responses to intruder/resident confrontations of golden hamsters during the rest period (ZT2) as measured by heart rate, core body temperature, and general activity were significantly stronger compared to stressor exposure during the activity time (ZT14).12
Although we and others began to study the interactions between the type and duration of stressors and the time of the day and their effects on parameters like HPA axis activity, physiological rhythms, or the immune status, the exact mechanisms underlying the diurnal differences in the stress response remain to be elucidated.
Can Stress Directly Affect the Central Clock?
GRs—A CHECKPOINT FO R THE INTERACTION BETWEEN GC AND THE CIRCADIAN SYSTEM AT THE PERIPHERAL LEVEL
Acute Stress and the Suprachiasmatic Nucleus
Chronic Stress and the Suprachiasmatic Nucleus
of the activity rhythm in mice.42 In contrast, a reduction in PER2 oscillation amplitude has been reported in the rat SCN following 4 weeks of chronic unpredictable stress.16 The same stress paradigm also led to a decreased CLOCK and BMAL1 protein expression in the central clock.17 Likewise, seven days of repeated restraint decreased the PER2 protein expression in the mouse SCN.20
Stress Effects on the Suprachiasmatic Nucleus Might be Dependent on the Time of Day
This truly indicates that a GC signal evoked by stressor exposure can be sensed by and perturb core clock components in the SCN, but only at certain times of the day. The time-dependent stress signal is likely perceived via intermediary GR-containing brain areas like the PVN, dorsomedial hypothalamic nucleus, or the raphe nuclei.27
Stress and Depressive-Like Behavior Might be Linked to the Circadian Clock
A link between circadian rhythm disturbances and mood disorders is beyond doubt. Virtually all of the successful treatments for mood disorders seem to affect circadian rhythms and it is assumed that stabilization and/or resetting of these rhythms by treatments are crucial for therapeutic efficacy (for review see Ref. 28). For instance, the effects of seasonal affective disorder, a mood disorder that correlates with the extremely shortened daily light period during the winter season, can be alleviated by bright light therapy. Patients suffering from seasonal affective disorder have abnormal levels of the dark phase hormone melatonin and exhibit a delayed chronobiological cycle.49 Since clock genes, especially Per1 and Per2, are inducible by light in the SCN, early morning light can phase-advance behavioral and endocrine rhythms, thus alleviating depressive-like symptoms.25
SUMMARY AND FUTURE DIRECTIONS
Vice versa, stress affects core clock components such as PER2 and CLOCK/BMAL1 within the central oscillator, which is often correlated with depressive-like behavior.
In addition, rotating shift-work and frequent jet lag are provoked by our modern-life habits. Individuals who are exposed to these lifestyles have a greater risk of developing both somatic and affective disorders. Human epidemiological studies have shown that rotating shift nurses exhibit a higher risk to suffer from breast cancer compared to day shift nurses.38 Likewise, shift-work experience is associated with a higher incidence of major depressive disorder.6
Given the interrelated influence of these bodily control systems, a proper functioning of the systems is critical for a healthy body and mind. It becomes clear that the balance between the two systems is crucial for the maintenance of proper circadian rhythms such as the GC rhythms that exert widespread function within our bodies.
Further studies are required to understand the exact molecular interactions between these two systems and their interplay in the development of human pathology to resolve the outstanding issues.
This Article was published in an Elsevier book.
References
- Balsalobre A, Brown SA, Marcacci L, et al. Resetting of circadian time in peripheral tissues by glucocorticoid signaling. Science. 2000;289:2344–2347.
- Bartlang MS, Neumann ID, Slattery DA, et al. Time matters: pathological effects of repeated psychosocial stress during the active, but not inactive, phase of male mice. J Endocrinol. 2012;215:425–437.
- Bartlang MS, Savelyev SA, Johansson AS, Reber SO, Helfrich-Forster C, Lundkvist GB. Repeated psychosocial stress at night, but not day, affects the central molecular clock. Chronobiol Int. 2014;31:996–1007.
- Cheifetz PN. The daily rhythm of the secretion of corticotrophin and corticosterone in rats and mice. J Endocrinol. 1971;49:xi–xii.
- Chrousos GP. Stress and disorders of the stress system. Nat Rev Endocrinol. 2009;5:374–381.
- Cole RJ, Loving RT, Kripke DF. Psychiatric aspects of shiftwork. Occup Med. 1990;5:301–314.
- Dibner C, Schibler U, Albrecht U. The mammalian circadian timing system: organization and coordination of central and peripheral clocks. Annu Rev Physiol. 2010;72:517–549.
- Dickmeis T. Glucocorticoids and the circadian clock. J Endocrinol. 2009;200:3–22.
- Dubovicky M, Mach M, Key M, Morris M, Paton S, Lucot JB. Diurnal behavioral and endocrine effects of chronic shaker stress in mice. Neuro Endocrinol Lett. 2007;28:846–853.
- Elenkov IJ, Wilder RL, Chrousos GP, Vizi ES. The sympathetic nerve – an integrative interface between two supersystems: the brain and the immune system. Pharmacol Rev. 2000;52:595–638.
- Engelmann M, Ebner K, Landgraf R, Wotjak CT. Swim stress triggers the release of vasopressin within the suprachiasmatic nucleus of male rats. Brain Res. 1998;792:343–347.
- Gattermann R, Weinandy R. Time of day and stress response to different stressors in experimental animals. Part I: golden hamster (Mesocricetus auratus Waterhouse, 1839). J Exp Anim Sci. 1996;38:66–76.
- Gorka Z, Moryl E, Papp M. Effect of chronic mild stress on circadian rhythms in the locomotor activity in rats. Pharmacol Biochem Behav. 1996;54:229–234.
- Guldner FH. Numbers of neurons and astroglial cells in the suprachiasmatic nucleus of male and female rats. Exp Brain Res. 1983;50:373–376.
- Hastings MH, Brancaccio M, Maywood ES. Circadian pacemaking in cells and circuits of the suprachiasmatic nucleus. J Neuroendocrinol. 2014;26:2–10.
- Jiang WG, Li SX, Zhou SJ, Sun Y, Shi J, Lu L. Chronic unpredictable stress induces a reversible change of PER2 rhythm in the suprachiasmatic nucleus. Brain Res. 2011;1399:25–32.
- Jiang WG, Li SX, Liu JF, et al. Hippocampal CLOCK protein participates in the persistence of depressive-like behavior induced by chronic unpredictable stress. Psychopharmacology. 2013;227:79–92.
- Kalsbeek A, Buijs RM. Output pathways of the mammalian suprachiasmatic nucleus: coding circadian time by transmitter selection and specific targeting. Cell Tissue Res. 2002;309:109–118.
- Kalsbeek A, van der Spek R, Lei J, Endert E, Buijs RM, Fliers E. Circadian rhythms in the hypothalamo-pituitary-adrenal (HPA) axis. Mol Cell Endocrinol. 2012;349:20–29.
- Kinoshita C, Miyazaki K, Ishida N. Chronic stress affects PERIOD2 expression through glycogen synthase kinase-3beta phosphorylation in the central clock. Neuroreport. 2012;23:98–102.
- Kolbe I, Dumbell R, Oster H. Circadian clocks and the interaction between stress axis and adipose function. Int J Endocrinol. 2015;2015:693204.
- Koresh O, Kozlovsky N, Kaplan Z, Zohar J, Matar MA, Cohen H. The long-term abnormalities in circadian expression of Period 1 and Period 2 genes in response to stress is normalized by agomelatine administered immediately after exposure. Eur Neuropsychopharmacol. 2012;22:205–221.
- Lamia KA, Papp SJ, Yu RT, et al. Cryptochromes mediate rhythmic repression of the glucocorticoid receptor. Nature. 2011;480:552–556.
- Larsen PJ, Vrang N, Moller M, et al. The diurnal expression of genes encoding vasopressin and vasoactive intestinal peptide within the rat suprachiasmatic nucleus is influenced by circulating glucocorticoids. Brain Res Mol Brain Res. 1994;27:342–346.
- Lewy AJ, Sack RL, Miller LS, Hoban TM. Antidepressant and circadian phase-shifting effects of light. Science. 1987;235:352–354.
- Lightman SL. The neuroendocrinology of stress: a never ending story. J Neuroendocrinol. 2008;20:880–884.
- Malek ZS, Sage D, Pevet P, Raison S. Daily rhythm of tryptophan hydroxylase-2 messenger ribonucleic acid within raphe neurons is induced by corticoid daily surge and modulated by enhanced locomotor activity. Endocrinology. 2007;148:5165–5172.
- McClung CA. Circadian genes, rhythms and the biology of mood disorders. Pharmacol Ther. 2007;114:222–232.
- Meerlo P, van den Hoofdakker RH, Koolhaas JM, Daan S. Stressinduced changes in circadian rhythms of body temperature and activity in rats are not caused by pacemaker changes. J Biol Rhythms. 1997;12:80–92.
- Meyer-Bernstein EL, Jetton AE, Matsumoto SI, Markuns JF, Lehman MN, Bittman EL. Effects of suprachiasmatic transplants on circadian rhythms of neuroendocrine function in golden hamsters. Endocrinology. 1999;140:207–218.
- Moore RY, Eichler VB. Loss of a circadian adrenal corticosterone rhythm following suprachiasmatic lesions in the rat. Brain Res. 1972;42:201–206.
- Moore RY. Entrainment pathways and the functional organization of the circadian system. Prog Brain Res. 1996;111:103–119.
- Moreau JL, Scherschlicht R, Jenck F, Martin JR. Chronic mild stress-induced anhedonia model of depression; sleep abnormalities and curative effects of electroshock treatment. Behav Pharmacol. 1995;6:682–687.
- Nader N, Chrousos GP, Kino T. Interactions of the circadian CLOCK system and the HPA axis. Trends Endocrinol Metab. 2010;21:277–286.
- Oster H, Damerow S, Kiessling S, et al. The circadian rhythm of glucocorticoids is regulated by a gating mechanism residing in the adrenal cortical clock. Cell Metab. 2006;4:163–173.
- Ratman D, Vanden Berghe W, Dejager L, et al. How glucocorticoid receptors modulate the activity of other transcription factors: a scope beyond tethering. Mol Cell Endocrinol. 2013;380:41–54.
- Reppert SM, Weaver DR. Coordination of circadian timing in mammals. Nature. 2002;418:935–941.
- Schernhammer ES, Laden F, Speizer FE, et al. Rotating night shifts and risk of breast cancer in women participating in the nurses’ health study. J Natl Cancer Inst. 2001;93:1563–1568.
- Selye H. Stress and the general adaptation syndrome. Br Med J. 1950;1:1383–1392.
- Silver R, LeSauter J, Tresco PA, Lehman MN. A diffusible coupling signal from the transplanted suprachiasmatic nucleus controlling circadian locomotor rhythms. Nature. 1996;382:810–813.
- So AY, Bernal TU, Pillsbury ML, Yamamoto KR, Feldman BJ. Glucocorticoid regulation of the circadian clock modulates glucose homeostasis. Proc Natl Acad Sci USA. 2009;106:17582–17587.
- Solberg LC, Horton TH, Turek FW. Circadian rhythms and depression: effects of exercise in an animal model. Am J Physiol. 1999;276:R152–R161.
- Son GH, Chung S, Choe HK, et al. Adrenal peripheral clock controls the autonomous circadian rhythm of glucocorticoid by causing rhythmic steroid production. Proc Natl Acad Sci USA. 2008;105:20970–20975.
- Stephan FK, Zucker I. Circadian rhythms in drinking behavior and locomotor activity of rats are eliminated by hypothalamic lesions. Proc Natl Acad Sci USA. 1972;69:1583–1586.
- Ulrich-Lai YM, Arnhold MM, Engeland WC. Adrenal splanchnic innervation contributes to the diurnal rhythm of plasma corticosterone in rats by modulating adrenal sensitivity to ACTH. Am J Physiol. 2006;290:R1128–R1135.
- Ushijima K, Morikawa T, To H, Higuchi S, Ohdo S. Chronobiological disturbances with hyperthermia and hypercortisolism induced by chronic mild stress in rats. Behav Brain Res. 2006;173:326–330.
- Weitzman ED, Fukushima D, Nogeire C, Roffwarg H, Gallagher TF, Hellman L. Twenty-four hour pattern of the episodic secretion of cortisol in normal subjects. J Clin Endocrinol Metab. 1971;33:14–22.
- Windle RJ, Wood SA, Shanks N, Lightman SL, Ingram CD. Ultradian rhythm of basal corticosterone release in the female rat: dynamic interaction with the response to acute stress. Endocrinology. 1998;139:443–450.
- Winkler D, Pjrek E, Praschak-Rieder N, et al. Actigraphy in patients with seasonal affective disorder and healthy control subjects treated with light therapy. Biol Psychiatry. 2005;58:331–336.
- Yamamoto T, Nakahata Y, Tanaka M, et al. Acute physical stress elevates mouse period1 mRNA expression in mouse peripheral tissues via a glucocorticoid-responsive element. J Biol Chem. 2005;280:42036–42043.
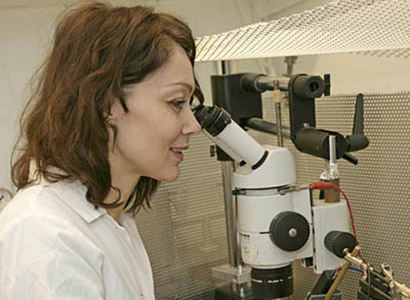